A Revolution in Biology - by Kasra - Bits of Wonder
This note was originally taken from: https://www.bitsofwonder.co/p/a-revolution-in-biology
Prelude: Michael Levin is a scientist at Tufts University who many have described as one of the most revolutionary biologists of our age. His work has been featured everywhere from Scientific American to the Lex Fridman podcast and The New Yorker. I spent the past month reading a bunch of his papers and interviews in an attempt to answer the question: what is Michael Levin on about and why does it matter? The essay below is the result of that investigation.
What are the biggest mysteries in science? When we ask this question we tend to talk about big picture topics like dark matter, consciousness, aliens, and parallel universes. We don’t talk as much about something that is more commonplace yet equally astonishing: how does the human body construct itself out of a single cell, like when a single fertilized egg cell develops into an embryo and ultimately into a fully-fledged adult?
We don’t tend to ask this question because we’re used to it—babies are born, acorns turn into trees, and eggs hatch into chickens every day. But there is indeed something perplexing about it. Think about what your cells have to do in the process of constructing your body: they have to coordinate their positions to follow a detailed architecture of bones, skin, muscles, and organs; they have to construct and wire together the hundred billion neurons of your brain; each cell has to decide what kind of cell to specialize into, and how much to duplicate to ensure all the proportions of your body parts are correct. How do so many individual units cooperate to self-assemble into a large, functional whole?
Well, let’s first consider a very different kind of functional whole: the computers, cars, and other machines that make up our built environment. These things are also complex functional wholes that are made up of countless tiny parts. But they’re “dead” in the sense that they don’t construct and heal themselves. The only thing that enables all the tiny parts to work together is the fact that we designed and put together all the parts ourselves, meticulously executing a detailed plan. The trouble with biology is that you don’t get this kind of top-down planning: when an egg cell proliferates into a full body, there is no “central command center” that has access to the entire developing body and can dictate what each part does. There is no brain around to tell the body how to construct the brain itself.
In the absence of top-down design, our conventional picture of biology is that everything happens in a bottom-up manner: molecular mechanisms dictate the functions of cells, which dictate the functions of your organs and which ultimately control your body. What is the thing at the very bottom of this hierarchy—the foundation for everything else in life? The genome. Genes are considered the fundamental code of life, so when it comes to figuring out questions of how the body develops, or how to cure diseases or change specific biological traits, we tend to look there. We spend much of our research efforts doing things like studying the gene that triggers the construction of eyes, or trying to identify the genetic underpinnings of Alzheimer’s and cancer, or studying how mutations in a specific gene alters a fruit fly’s circadian rhythm Ever since we finished sequencing the human genome back in 2003, we’ve been inundated by genomic data that we don’t quite know what to do with, but the emphasis is primarily on how genes and chemical pathways determine the high-level structure of the organism.1
That is, until Michael Levin (and many others) entered the scene. They came in and said: genes are great, and they do contain much of the necessary information for building our bodies. But they don’t contain all of it, and they are not always a useful level of abstraction of understanding how the body develops, and consequently they are not always the best way to intervene with biology (e.g. to regenerate damaged organs, or to cure diseases like cancer). If you’ve ever done any programming, you know that there are many levels of abstraction—higher-level and lower-level programming languages, higher-level and lower-level API’s—at which you can try to understand or manipulate the software that runs in your computer. Levin’s point is that genes are like machine code, and modern-day programmers never think about machine code—they think about higher-level software constructs like objects, modules, and applications. The bold claim embedded in his work—the real revolution here—is that higher levels of abstraction and control meaningfully exist in biology. And one of the ways in which this higher level of abstraction manifests is in something called the bioelectric network of the organism.2
We usually think of neurons as the only cells in our body that produce intelligent behavior by communicating in large networks. Neurons are constantly communicating with each other in the form of electrical patterns on their membrane and neurotransmitters, which are chemicals that transfer messages between cells. But it turns out that cells throughout the body have the exact same building blocks for such communication. They do the same communication, but slower. Levin and company call this the bioelectric network, as distinguished from a neural network.
In the past few decades we’ve discovered all the ways in which bioelectric networks distributed through the body do the same kinds of things that brains do: store memories, solve problems, and guide development. To get a sense of the bioelectric network in action, we have to talk about a mind-blowing creature called the planarian. This little critter (about 2cm in length) is a developmental “genius” of sorts: it doesn’t age, it doesn’t get cancer, and it is extremely regenerative, capable of regenerating any part of its body that gets cut off, even if it’s cut up into more than 250 pieces.
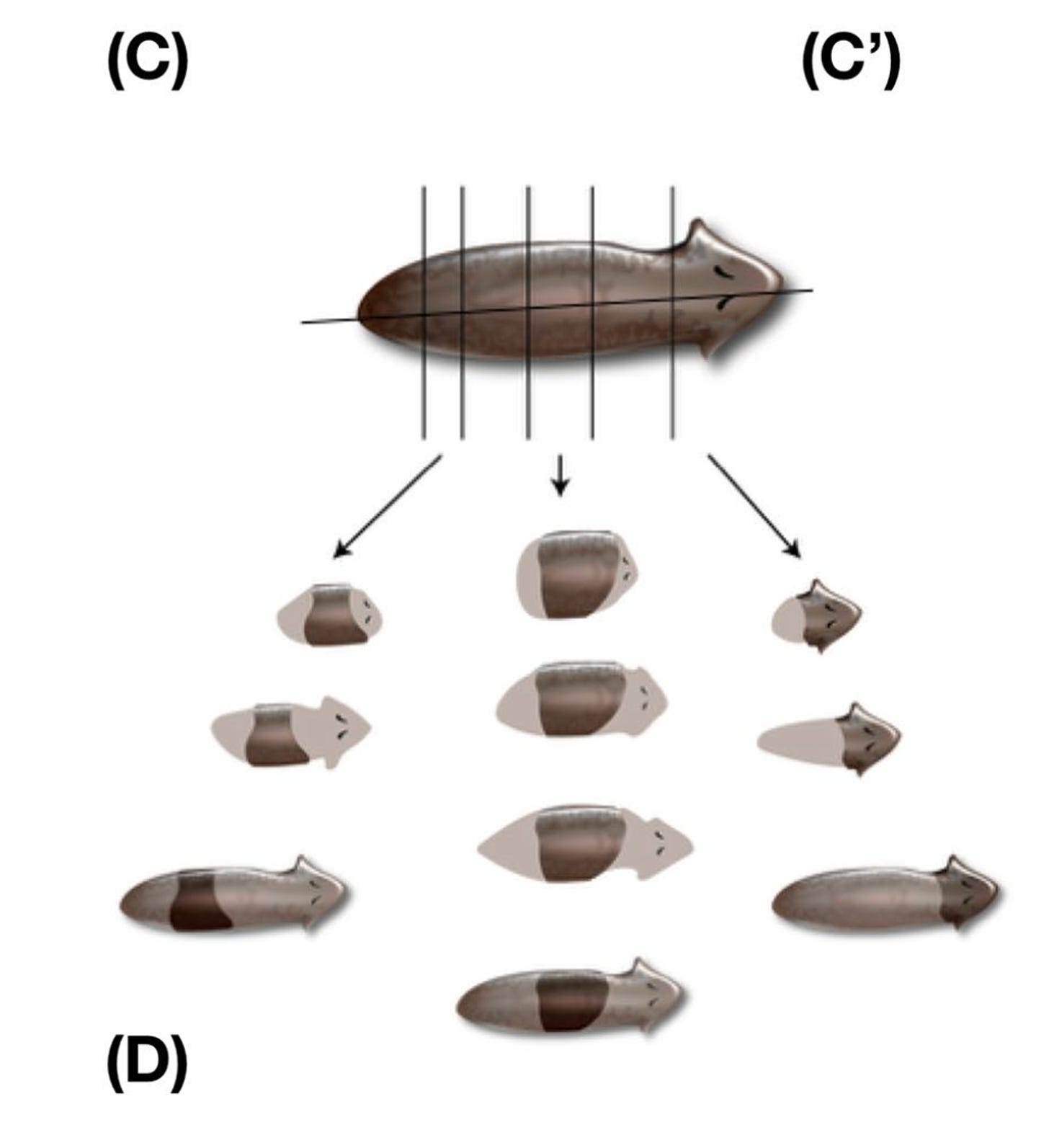
Diagram depicting the planarian’s ability to regrow even when cut into many pieces, from this paper.
The question we asked earlier—how an egg cell expands into a full body—applies equally to how the planarian regenerates its entire body from just a tiny piece of tissue. (We can think of development itself as a kind of regeneration event.) While there’s still much to understand about the planarian’s profound regenerative qualities, Levin and others have shown that the bioelectric network of the worm plays a crucial part.3
Imagine taking one of these worms and splitting it into two. You now have two half-worms, and each of those half-worms is tasked with rebuilding the rest of its body. There’s a crucial decision here that the cells have to make: what part of the body do we already have, and what part do we need to build? One of the half-worms needs to produce a tail, and the other half-worm needs to produce a head. But the cells are at the very middle of the body, extremely far (from a cell’s perspective) from both the head and the tail. How do the cells have any idea what they should generate?
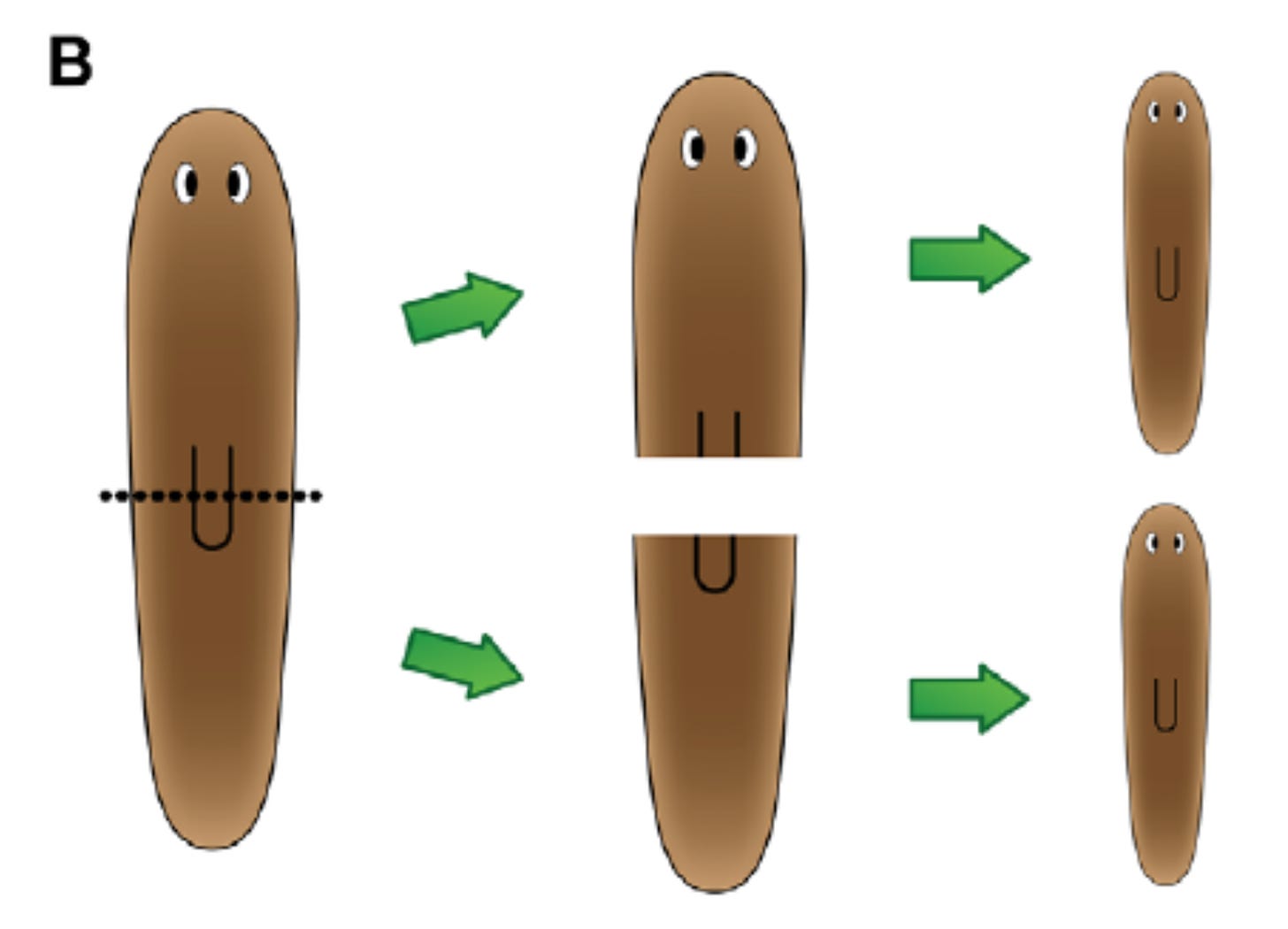
When the planarian is divided into two, cells on either side of its cut have to form the missing half of the body. From this paper.
The answer, at least in part, is that all along the body the cells of the worm have a gradient of “resting membrane potentials”, which is effectively a stable electrical state. The cells keep track of their “position” in the body in this way, and experiments have demonstrated that the cell’s electrical state relative to the rest of the body is what determines whether it will proliferate into a head or a tail.4
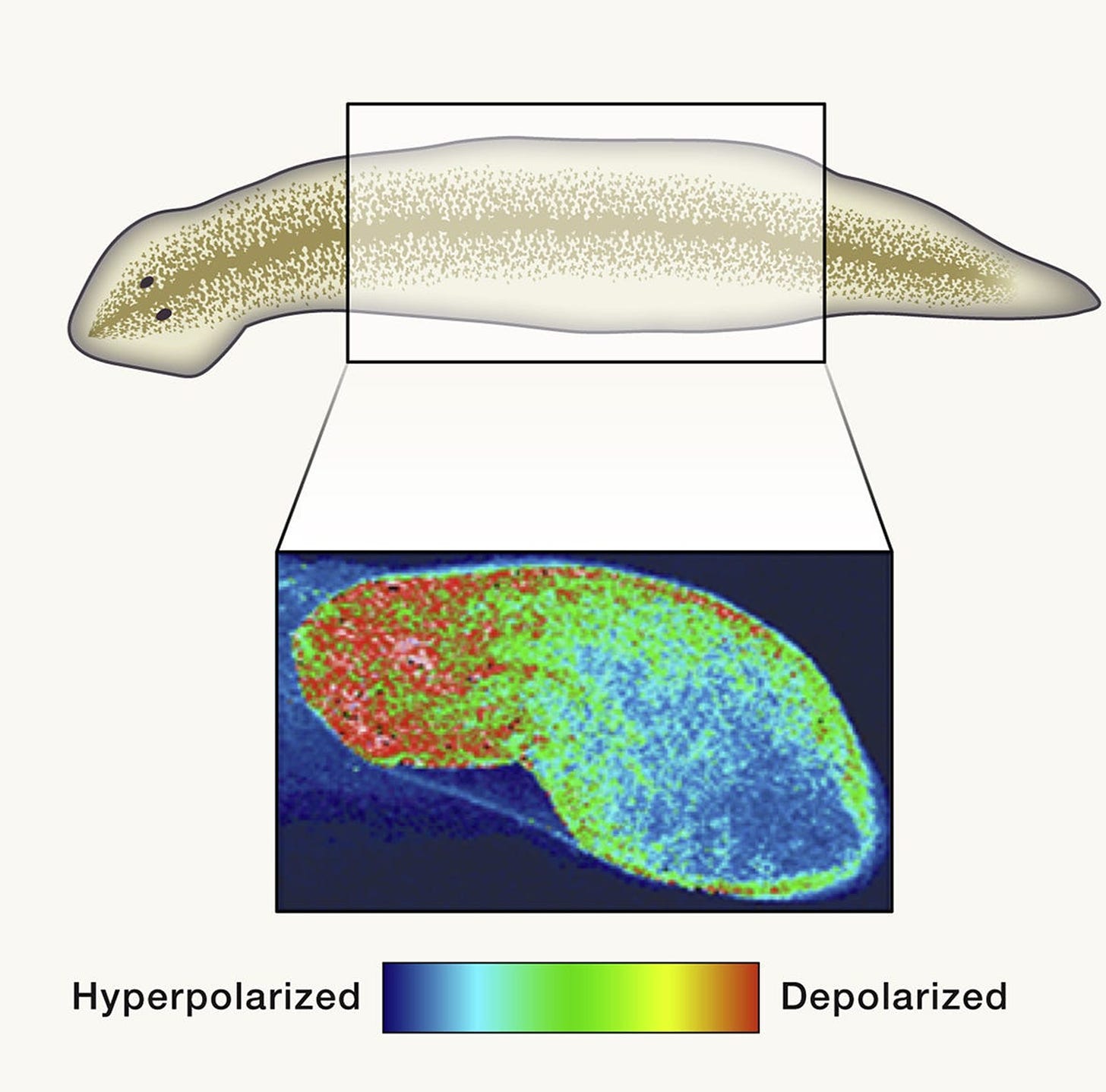
A picture of the bioelectric network of the worm (taken from this post). If you measure the electrical potential all along its body, you see this gradient from its head to its tail.
Why does all this matter? Because once we understand how cells coordinate to decide what parts of the body to build, we can begin to intervene with that development to create new body structures. And they’ve done exactly that: Levin’s team was able to induce the worm to generate two heads instead of one head, by putting it into a solution of drugs that blocked specific ion channels (which in turn altered the electrical state of the cells). They’ve also induced the worm to generate no heads at all, or to generate the head of a different worm species. All of these are living, functional worms, just with a very different body structure.
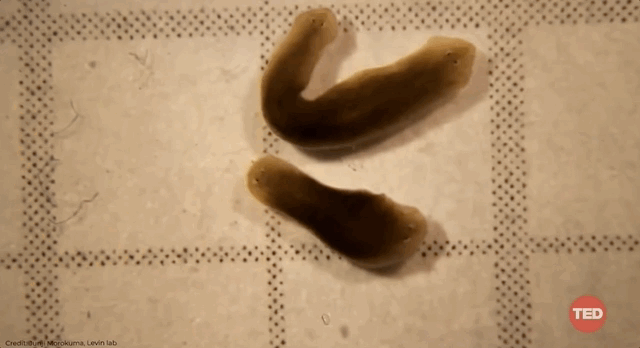
Video of two-headed worms produced in Levin’s lab floating around, taken from this video.
Keep in mind a crucial point: in all these experiments, the genes of the worms are never edited. You get a wildly different functional worm with the same genes. And what’s even wilder is that some of these changes are enduring: without any further drugs or modifications, the two-headed worm produces offspring that are also two-headed, indefinitely.5 Think about what this means: we’ve achieved a permanent change in the structure of the worm, without changing its genes. We have transcended the genetic code and are instead learning to crack the bioelectric code of the body.
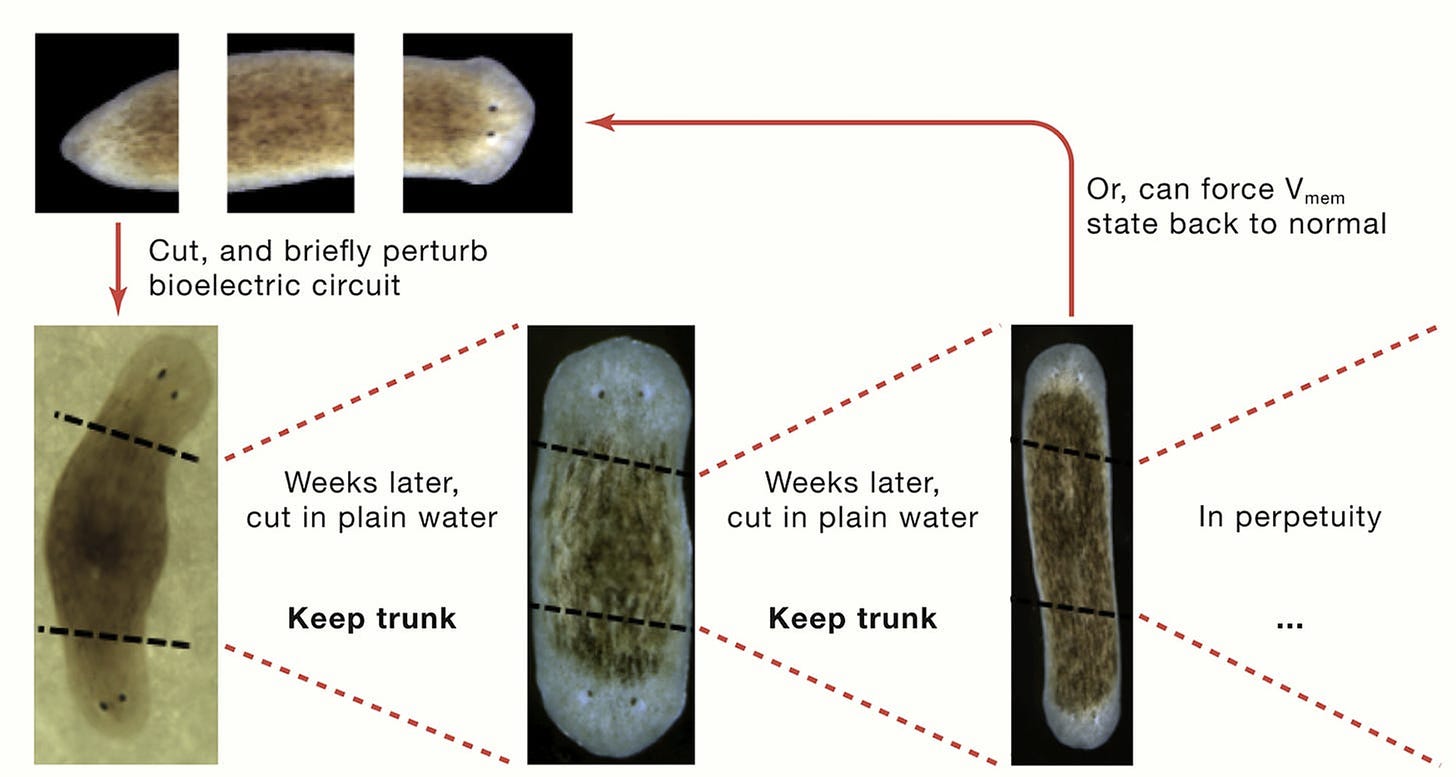
The two-headed worms continue to reproduce as two-headed worms indefinitely (taken from this post).
The worm is just one example: Levin’s lab and others have already demonstrated an astonishing level of control over development by modulating bioelectric networks. They’ve done things like getting frogs to develop extra limbs, and getting them to develop an eye in their gut, or an eye in their tail that they can actually see out of. The end goal that Levin dreams of is an “anatomical compiler” – a program which takes as input a specification for an arbitrary organ or body plan, and outputs the specific set of chemical and electrical signals needed to generate that organ. Imagine 3-d printing entire synthetic organs and organisms, except instead of having to specify all the micro-level details, you can just give a high-level description like “an extra eye at the tail.” This is Dall-E but for biology. And in the very long run, it could be the answer to virtually all of biomedicine, including traumatic injury, birth defects, degenerative disease, cancer, and aging.6
So on a practical level, the impact of Levin’s work is a shift away from genes as the only determinant of structure, shifting instead towards the bioelectric network. But there’s a broader thesis here, which is recognizing that the terms “intelligence” and “cognition” apply to much more of biology than we tend to think. The very process of development has an intelligence of its own: for example, if you take a tadpole (the precursor to a frog), and manually scramble its facial organs, those facial organs will relocate back to the correct place as the tadpole matures.
The developing body of the tadpole is actively moving towards a “goal” state: it’s not a hard-coded system that blindly follows a predetermined set of steps encoded in the genes. The manual scrambling the experimenters created (called “picasso frogs”) is a situation that would not have occurred in the evolutionary environment, so it can’t be something it was specifically selected for.
There are many more examples of such adaptability in biological systems, found at the level of individual cells and groups of cells. Levin defines “intelligence” as the capacity to achieve the same goal via different means, and over the years he and others have documented case after case of such adaptability. If a developing embryo is surgically cut into two, it develops into two separate, healthy twins rather than two half-bodies; the embryos restructure themselves on-the-fly in response to external perturbations. If a newt salamander’s cells are artificially enlarged, the tubules in its kidneys still develop to be the same objective size, by simply using fewer cells per tube. It’s like the cells are cooperating to produce a fixed tube size no matter how large the individual cells are. As the individual cells are made to be bigger and bigger, the salamander even develops tubules out of a single cell that turns in on itself:
So we have all these examples of fractal intelligence, at layers up and down the biology stack. But the profound flip-side of intelligence is creativity: biology is capable of not just recovering the same functionality when perturbed, but of adopting entirely new kinds of functionality when given the right set of prompts. Levin’s team has taken skin cells from an embryonic frog, and given them certain signals to create “biobots” that move on their own and even self-replicate. (Again: no genetic modifications, just taking ordinary stem cells and giving them drugs.) More recently they’ve taken cells from adult human lung tissue, and used them to construct moving biobots that can heal damaged neurons. There’s a whole world of latent creativity to be discovered at all levels of biology, and the potential applications are endless. Imagine creating little biobots that can attack cancerous cells, or clean up toxins in the environment, or heal degenerated nervous tissue.
The bigger, mind-blowing perspective shift in Levin’s work is to rethink what we think counts as an “agent”, with “goals” that it pursues. Is a cell an agent? An embryo? What about a liver? What about your immune system? Levin hypothesizes that way before evolution discovered agency and intelligent information-processing in brains, it had already discovered it in lower-level systems—in morphogenesis (the process by which an organism develops its high-level structure), in bacterial colonies, and even in networks of genes. These are systems that we have trouble viewing as autonomous entities capable of forming memories or having goals. But what I think is truly special about Levin’s work is that he doesn’t just advocate a shift in perspective from an armchair: his team has been putting in decades of experimental work demonstrating the validity and promise of these ideas. His focus has been not just on philosophizing about what is intelligence and agency, but instead asking: what definitions, what frameworks, will lead to the most fruitful lines of empirical research and potential applications? Once we view cells and groups of cells as having an innate intelligence, we can leverage that intelligence to our own ends.
It’s not just biomedicine that stands to gain from this perspective shift: if we think of our brains, our organs, and our cells as all having the same basic building blocks for cognition, then we can share tools and ideas from across fields. The notion of “cognitive science” expands beyond just the study of neurons in the brain, to any cell type that coordinates together, or really any collective at all, including groups of humans. Specific parallels have already been studied, like studying cancer as a “dissociative identity disorder” of cell groups, or the finding that ant colonies succumb to “visual illusions” of the same kind that brains do. Levin says that all intelligence is collective intelligence: all of these “diverse intelligences” are ultimately made up of parts, a large number of subunits that have their own competencies and lower-level intelligence, combining to produce something greater than the whole. We tend to think of ourselves as individuals, as an indivisible unit, but the same “collective intelligence” label applies to each one of us: you are ultimately a collection of a hundred billion neurons (and trillions of other cells) cooperating together, each cell having its own competencies and subgoals that sometimes even conflict. It's strange to think of ourselves in this way, our brains being on the same spectrum as a colony of ants or a flock of geese, but once you look into the details of how we function it's hard to see it any other way. Just as the human world is a society of selves, your body is a society of cells. The connection might be more than mere metaphor.
Credit to Suzanne for feedback on earlier drafts and this post for originally introducing me to Levin’s work.